Overview: Exotic phases of interacting flatland electron systems
One of the simplest ways to place electrons in a flatland is to confine them to the interface between two semiconductors with different bandgaps. Examples are shown in Fig. 1 where a “2D electron system” is formed at the interface between undoped GaAs and AlGaAs, or in a GaAs quantum well flanked by AlGaAs barriers. The larger bandgap of AlGaAs leads to its conduction-band energy (ECB) being higher than GaAs. The system is “modulation-doped” meaning that the dopant atoms (in this case, Si donors) are placed in AlGaAs at some distance away from the interface. The electrons from the donors find it energetically favorable to transfer to the GaAs layer where their energy is lower.
Now, the electrons in such a “flatland” are amazing! Because the electrons are spatially separated from the dopant atoms, scattering by the ionized impurities is immensely reduced. Thanks to the reduced disorder and scattering, such “clean” structures provide nearly ideal systems for studies of electron-electron interaction phenomena, especially at low temperatures and high magnetic fields where the thermal and kinetic energies of the electrons are quenched. The dominant electron interaction leads to various fascinating and exotic ground states that are often unexpected (see Fig. 2 for examples). Studies of such states are at the forefront of condensed matter research. They could lead to a better fundamental understanding of interaction phenomena, and they might help with advances in new concepts such as topological quantum computing. Our research is devoted to the materials science and physics of flatland electrons.
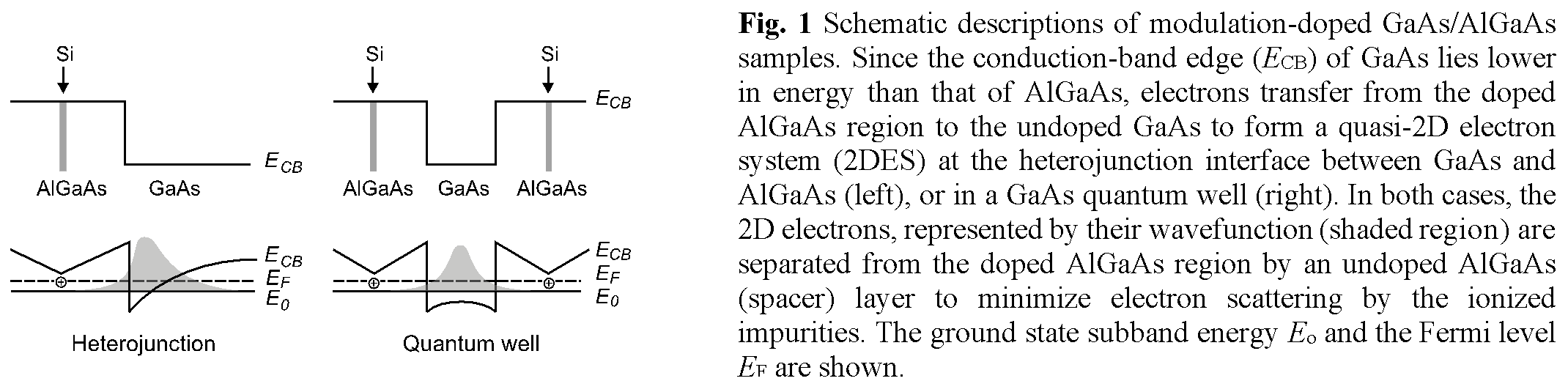
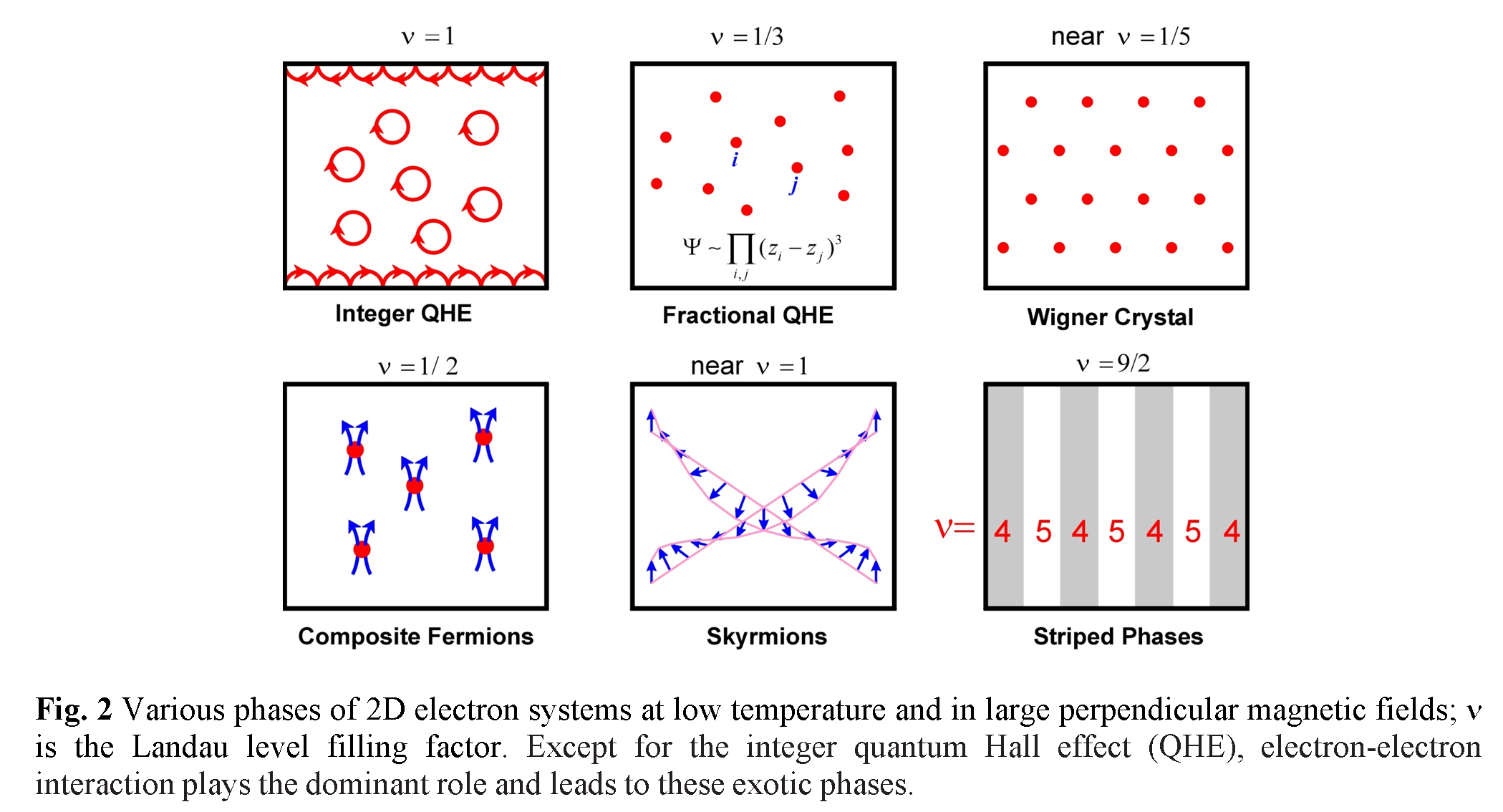
[Ref.: M. Shayegan, “Flatland Electrons in High Magnetic Fields,” book chapter in "High Magnetic Fields: Science and Technology," Vol. 3, edited by Fritz Herlach and Noboru Miura, (World Scientific, Singapore, 2006), pp. 31-60. (cond-mat/0505520)]
Fabrication of ultra-pure, thin-layer GaAs/AlAs structures via molecular beam epitaxy
Work in close collaboration with Dr. Loren Pfeiffer
A cornerstone of our research is the fabrication of selectively-doped, but otherwise pure, semiconductor structures where the carriers are spatially separated from the dopant atoms to reduce the ionized impurity scattering. The low-temperature mobility of the carriers in these structures far exceeds the values typically observed in the uniformly-doped materials, and can reach up to ~4 x 107 cm2/Vs. Thanks to the reduced disorder and scattering, they provide nearly ideal, "clean" systems for two of the most exciting and currently active areas of solid state physics and device research. One is the ballistic and quantum (wave) transport phenomena whose study is made possible by the long carrier mean-free-path and phase coherence length in such materials. With the help of high-resolution lithographic and novel growth techniques to produce low-dimensional structures, a wealth of new, albeit often single-body, mesoscopic phenomena and novel device concepts has emerged in recent years. The other area of research afforded by these materials deals with the fascinating and often unexpected electron states arising from the strong electron-electron interaction. Our research includes studies in both of these fields.
We have a very strong and close collaboration with Dr. Loren Pfeiffer, a world renown expert in the fabrication of GaAs/AlAs materials with the highest purity through molecular beam epitaxy (see figure). For more details, please see: Dr. Loren Pfeiffer
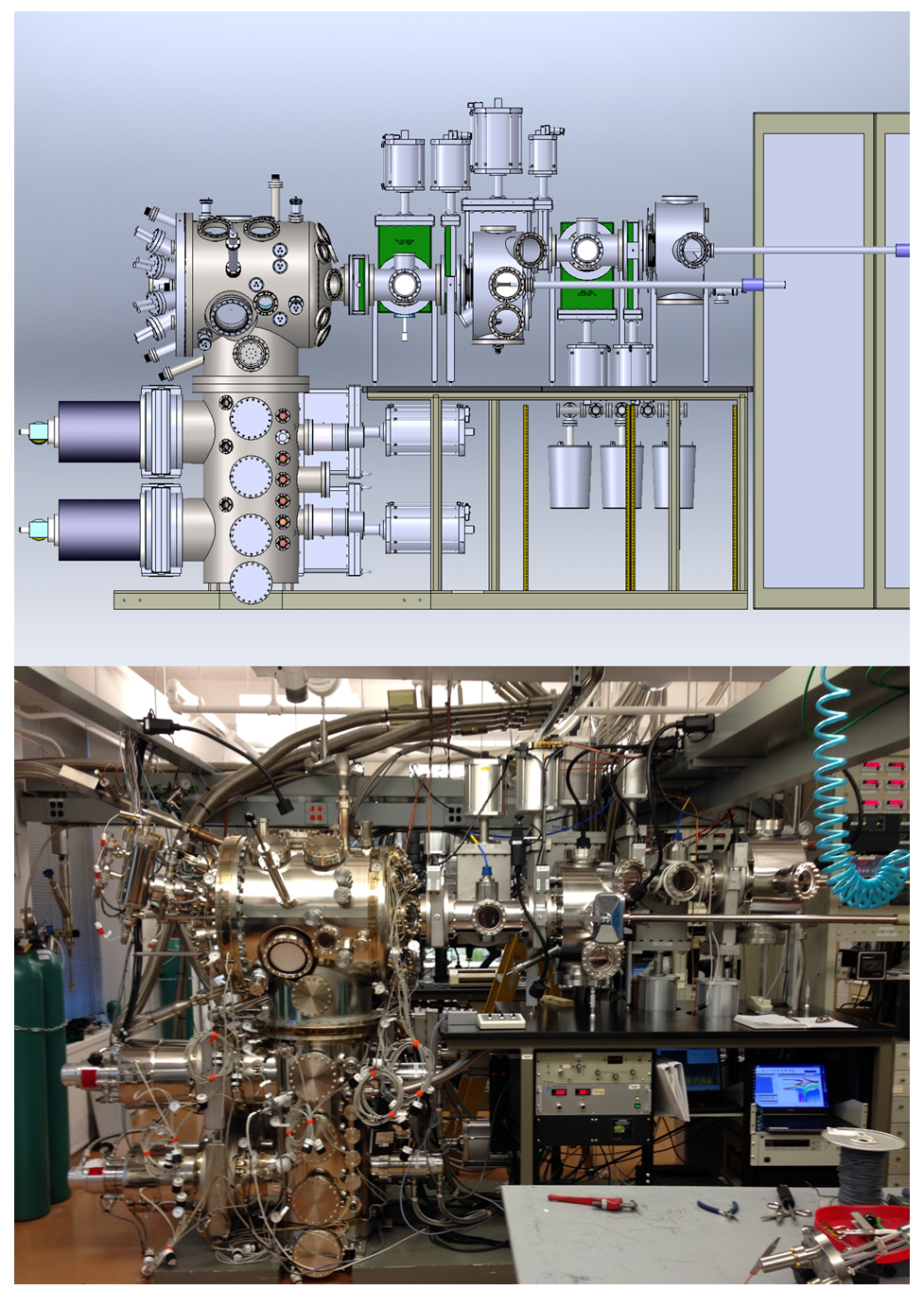
Two-dimensional electrons in AlAs quantum wells: a system with a “valley” degree of freedom
In the vast majority of GaAs/AlAs modulation-doped structures the electrons are confined in GaAs while AlAs, or more commonly, AlGaAs, is used as the barrier material. In such structures the electrons occupy the GaAs conduction band minimum at the ? point of the Brillouin zone (Fig. 1(a)). However, in a properly designed structure, one can confine electrons to the AlAs layer. In this case, the confined electrons occupy the energy band minima or “valleys” at the X points of the Brillouin zone Fig. 2(b). The ensuing AlAs 2D electron system has parameters that are very different from those of the standard 2D electrons in GaAs: the electrons have a much heavier effective mass which is also anisotropic, a much larger effective g-factor, and they occupy multiple conduction band valleys. Moreover, the valley occupation can be controlled via applying in-plane strain, thus changing the electronic properties of the 2D system qualitatively. Such control over the electronic properties through the manipulation of the valley degree of freedom renders the AlAs 2D electron system a prime candidate for studies of “valleytronic” devices and concepts. Indeed, our group was the first to demonstrate such manipulation, and suggested that it could be used to make functional devices or serve as a qubit for quantum computation. [Refs: Gunawan, Phys. Rev. Lett. 97, 186404 (2006); Phys. Rev. B 74, 155436 (2006)]
Recently we were able to improve the quality of the 2D electrons in AlAs tremendously, achieving mobilities exceeding 2x106 cm2/Vs. Figure 2 provides an example of the fractional quantum Hall data in such high mobility samples. Studies of the physics of these exotic 2D electron systems continue in our group. [Ref.: Y-J. Chung, Phys. Rev. Materials (Rapid Communication) 2, 071001(R) (2018)]
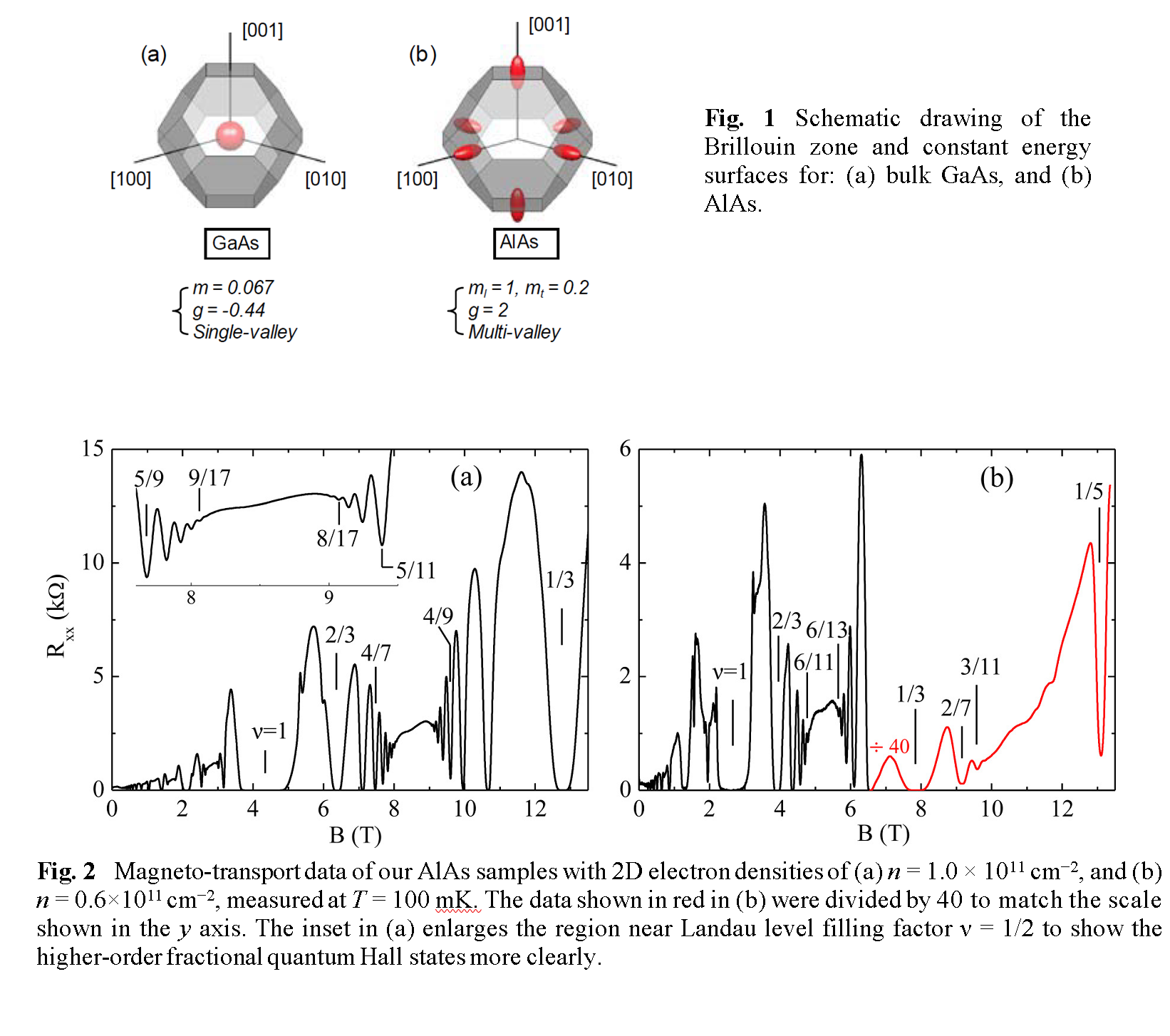
Composite fermions waltz to the tune of a Wigner crystal!
When the kinetic energy of a 2D electron system is quenched at very high perpendicular magnetic fields so that the Coulomb repulsion dominates, the electrons are expected to condense into an ordered array, forming a quantum Wigner crystal (WC). Although this exotic state has long been suspected in high-mobility 2D electrons at very low Landau level fillings (˝ « 1), its direct observation has been elusive. In our work we introduced a new technique and obtained experimental results directly probing the magnetic-field-induced WC. We measured the magneto-resistance of a bilayer electron system where one layer has a very low density and is in the WC regime (˝ « 1), while the other (“probe”) layer is near ˝ = 1/2 and hosts a sea of composite fermions (CFs) (Fig. 1). The data exhibit commensurability oscillations in the magneto-resistance of the CF layer, induced by the periodic potential of WC electrons in the other layer (Fig. 1), and provide a unique, direct glimpse at the symmetry of the WC, its lattice constant, and melting. They also demonstrate a striking example of how one can probe an exotic many-body state of 2D electrons using equally exotic quasi-particles of another many-body state.
Our latest results, from experiments done in collaboration with A. Hatke and L.W. Engel at the National High Magnetic Field Laboratory in Tallahassee, FL, reveal that the WC electrons return the favor! The data show that the pinning modes of the collective vibrations of the WC are affected by the fractional quantum Hall states of the CFs in the majority layer. [Refs.: Deng, Phys. Rev. Lett. 117, 096601 (2016), Featured in Physics, Editor’s Suggestion; Jo, Phys. Rev. Lett. 120, 016803 (2018), Editor’s Suggestion; Hattke, pre-print (2018)]
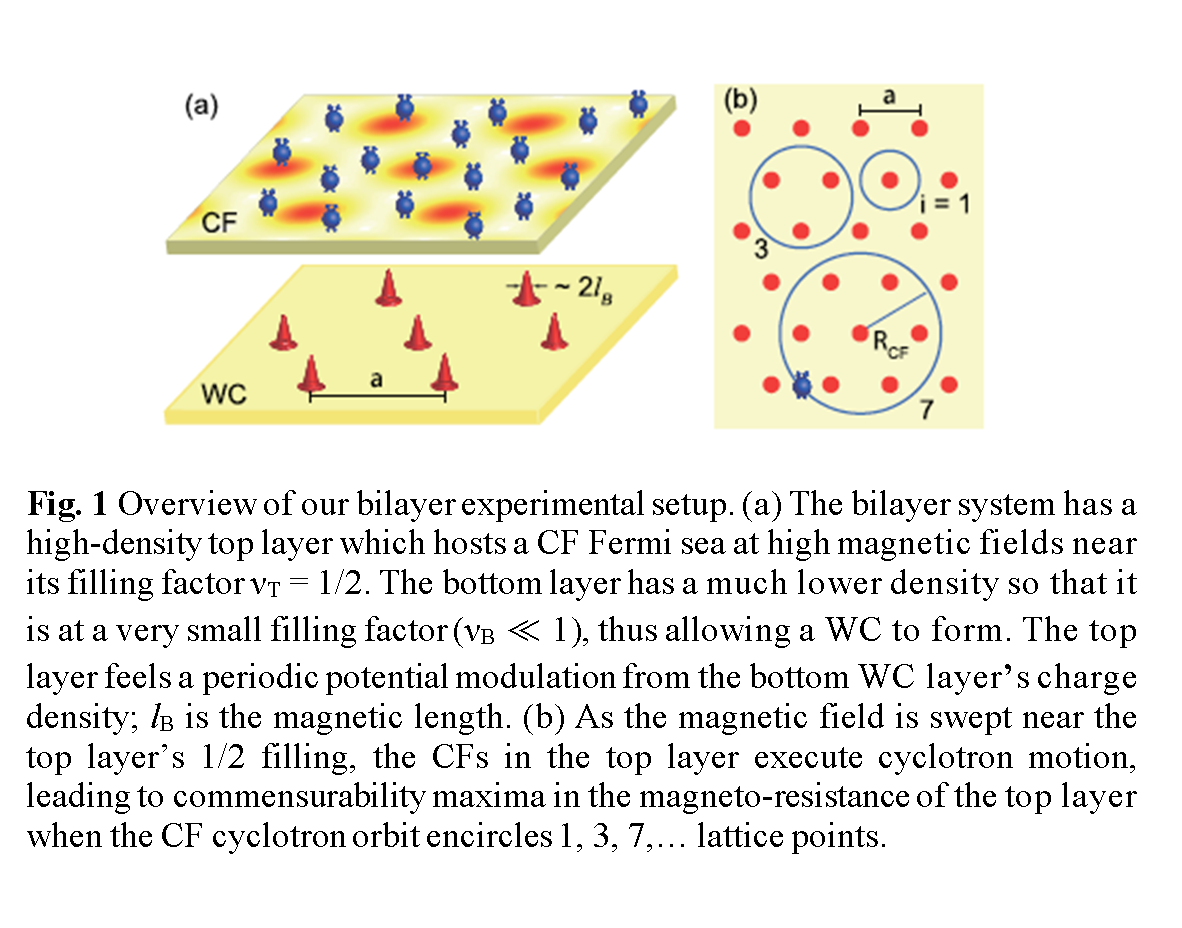
Signatures of an annular Fermi sea in 2D hole systems
The concept of a Fermi surface, the constant-energy surface containing all the occupied electron states in momentum, or wave vector, space plays a key role in determining electronic properties of conductors. In 2D carrier systems, the Fermi surface becomes a contour which, in the simplest case, encircles the occupied states. In this case, the area enclosed by the contour, which we refer to as the Fermi sea, is a simple disk. In general, however, the Fermi sea can have unusual shapes (Fig. 1). In our study, we reported the observation of a Fermi sea with a new topology, namely, one in the shape of an annulus (Fig. 2). Such a Fermi sea is expected in a variety of 2D systems where the energy band dispersion has a “Mexican hat” shape and supports a ring of extrema at finite k, but its experimental observation has been elusive. Our study provided: (i) theoretical evidence for the presence of an annular Fermi sea for the excited subband of 2D hole systems confined to wide GaAs quantum wells. and (ii) experimental signatures of the onset of its occupation as an abrupt rise in the sample resistance, accompanied by a sudden appearance of Shubnikov–de Haas oscillations at an unexpectedly high frequency whose value does not simply correspond to the (negligible) density of holes contained within the annular Fermi sea.
[Ref.: Jo, Phys. Rev. B 95, 035103 (2017)]
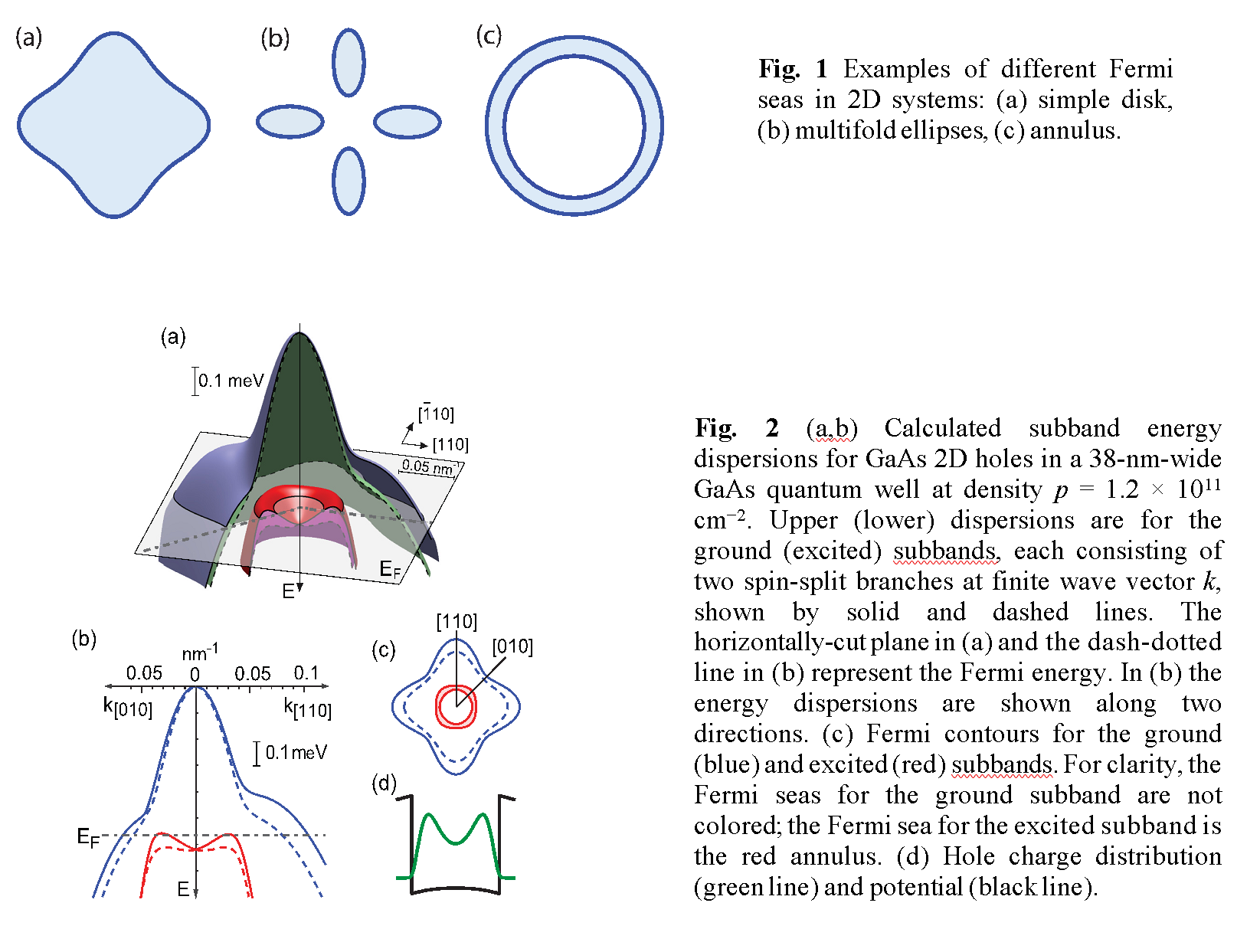
Tuning and probing Fermi contours of 2D holes via the application of uniaxial strain
The energy vs wave vector dispersions of GaAs 2D hole systems are complex. One goal of our research has been to quantitatively probe these dispersions, both experimentally and theoretically. Experimental determination of the 2D hole dispersions has long been a subject of interest. It continues to be a subject of active research, thanks partly to the interest in spintronic devices and also the possibility that holes in confined structures might have a long spin coherence time (because of the lack of overlap of holes’ p-type wave function with the nuclei) and be useful in quantum computing. We focused our efforts on tuning and probing the 2D holes’ dispersions using uniaxial strain in the 2D plane.
Figure 1 provides an example of the tunability, as well as complexity, of the 2D hole bands. Here we show the results of self-consistent calculations for the Fermi contours of 2D holes confined to a (001) GaAs square QW of width 17.5 nm, and subjected to uniaxial, in-plane strain (µ). The splitting between the two p+ and p- contours as well as the anisotropy of these contours is clear in Fig. 1 plots. As is evident in Fig. 1(a), the Fermi contours and the corresponding trajectories of 2D holes become significantly anisotropic for minute values of µ, of the order of 10-4 (see Fig. 1(a)). Our goal was to experimentally tune the anisotropy and measure it. We glued the sample to one side of a stacked piezo-actuator whose shape can be tuned via applying voltage (VP) to its leads, thus exerting the strain to the sample (Fig. 1(b)). To monitor the Fermi contour, we made samples with surface gratings that lead to a unidirectional, periodic potential (density) modulation for the 2DHS (Fig. 1(c)). The samples allowed us to observe ballistic commensurability (also known as Weiss) oscillations, namely, oscillations in the magneto-resistance resulting from the matching of the classical hole cyclotron orbit diameter with integer multiples of the period of the potential modulation (Fig. 1(c)). From the commensurability oscillations, we determined the shape and anisotropy of the ballistic cyclotron orbits and Fermi contours, and found them to be in quantitative agreement with the results of calculations shown in Fig. 1(a), although we could not resolve the spin-orbit split, p+ and p- spin subbands.
[Ref.: Jo, Appl. Phys. Lett. 110, 252103 (2017)]
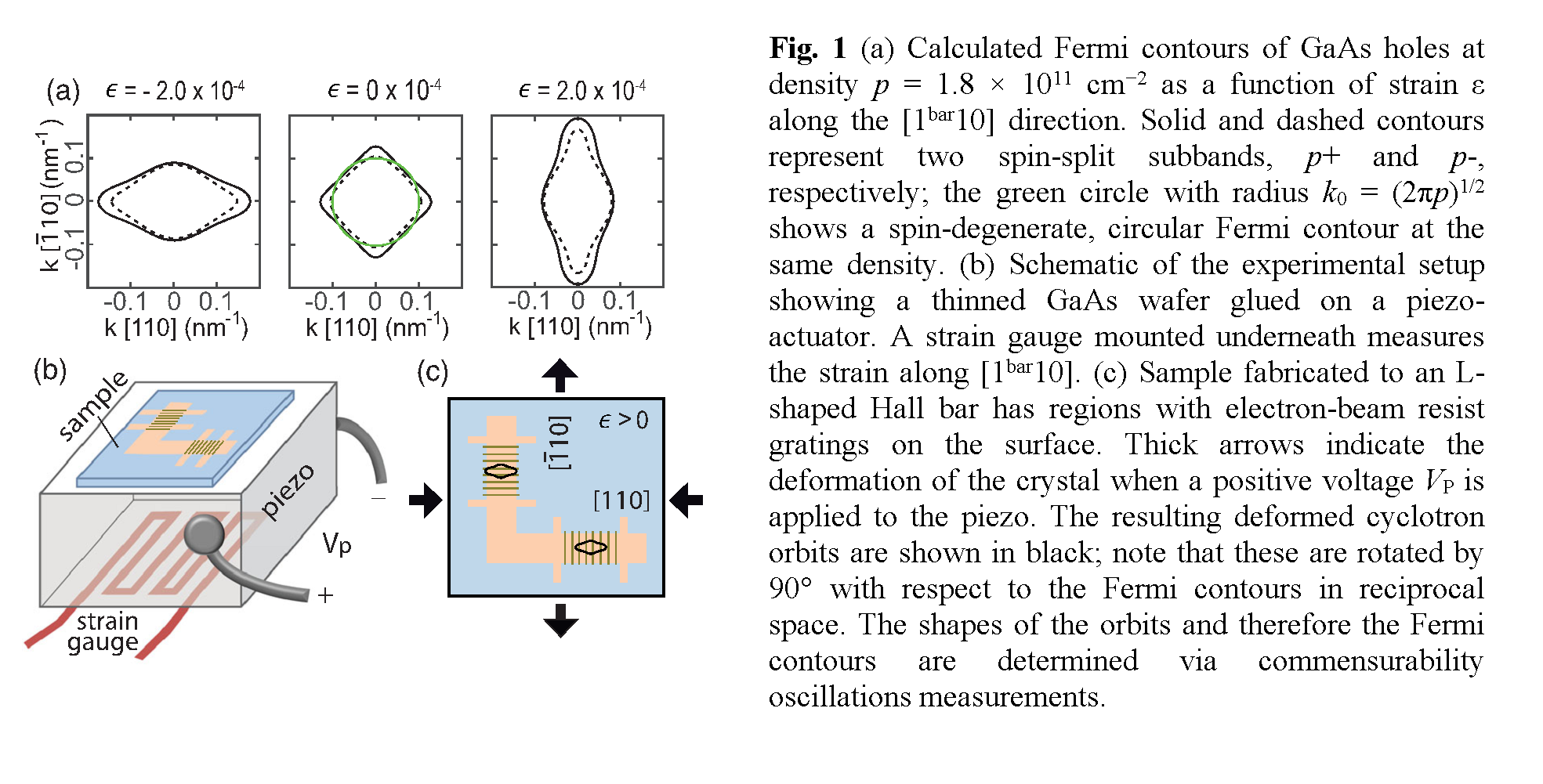
Transference of Fermi contour anisotropy to hole-flux composite fermions
There has been a surge of recent interest in the role of anisotropy in interaction-induced phenomena in 2D, charged, carrier systems. A fundamental question is how an anisotropy in the energy-band structure of the carriers at zero magnetic field affects the properties of the interacting particles at high fields, in particular of the composite fermions (CFs) and the fractional quantum Hall states (FQHSs). The CF formalism provides an extremely powerful yet very simple description of the interacting particles at high perpendicular magnetic fields. In the CF picture, an even number of flux quanta pair up with each carrier at high to form quasi-particles which, at Landau level filling factor ˝ = ˝, occupy a Fermi sea with a well-defined Fermi contour (Fig. 1). The existence of a CF Fermi contour raises the question whether fermionization preserves any low-field Fermi contour anisotropy (Fig. 1). This question is of particular importance in light of the intense recent interest in how anisotropy affects interacting carrier systems.
To answer this fundamental question we determined, via measurements of commensurability oscillations, the shape of the hole-flux CF Fermi contour near ˝ = ˝ as a function of strain. This challenging experiment required samples containing extremely high-quality 2D holes, as well as a very gentle and well-ordered periodic potential. We measured the magneto-resistance along two perpendicular arms of an L-shaped Hall bar, shown at different values of strain. The strain shifts the positions of the resistance minima observed on the flanks of ˝ = ˝ when the CF Fermi cyclotron orbit diameter becomes commensurate with the period of the periodic potential. For strains on the order of 10-4 we observed significant deformations of the shapes of the Fermi contours for both holes and CFs. Quantifying the anisotropy by the ratio (±) of the Fermi wave vectors along the principle axes of the Fermi contours, as shown in Fig. 2, our measurements showed that the Fermi contour anisotropy for CFs (±CF) is less than the anisotropy of their low-field hole (fermion) counterparts (&alphaF), and closely follows a simple relation &alfaCF = (±F)1/2. The energy gap measured for the ˝ = 2/3 FQHS, on the other hand, is nearly unaffected by the Fermi contour anisotropy up to ±F ~ 3.3, the highest anisotropy achieved in our experiments. These are fascinating results, and are finding verification in numerical calculations.
[Ref.: Jo, Phys. Rev. Lett. 119, 016402 (2017)]
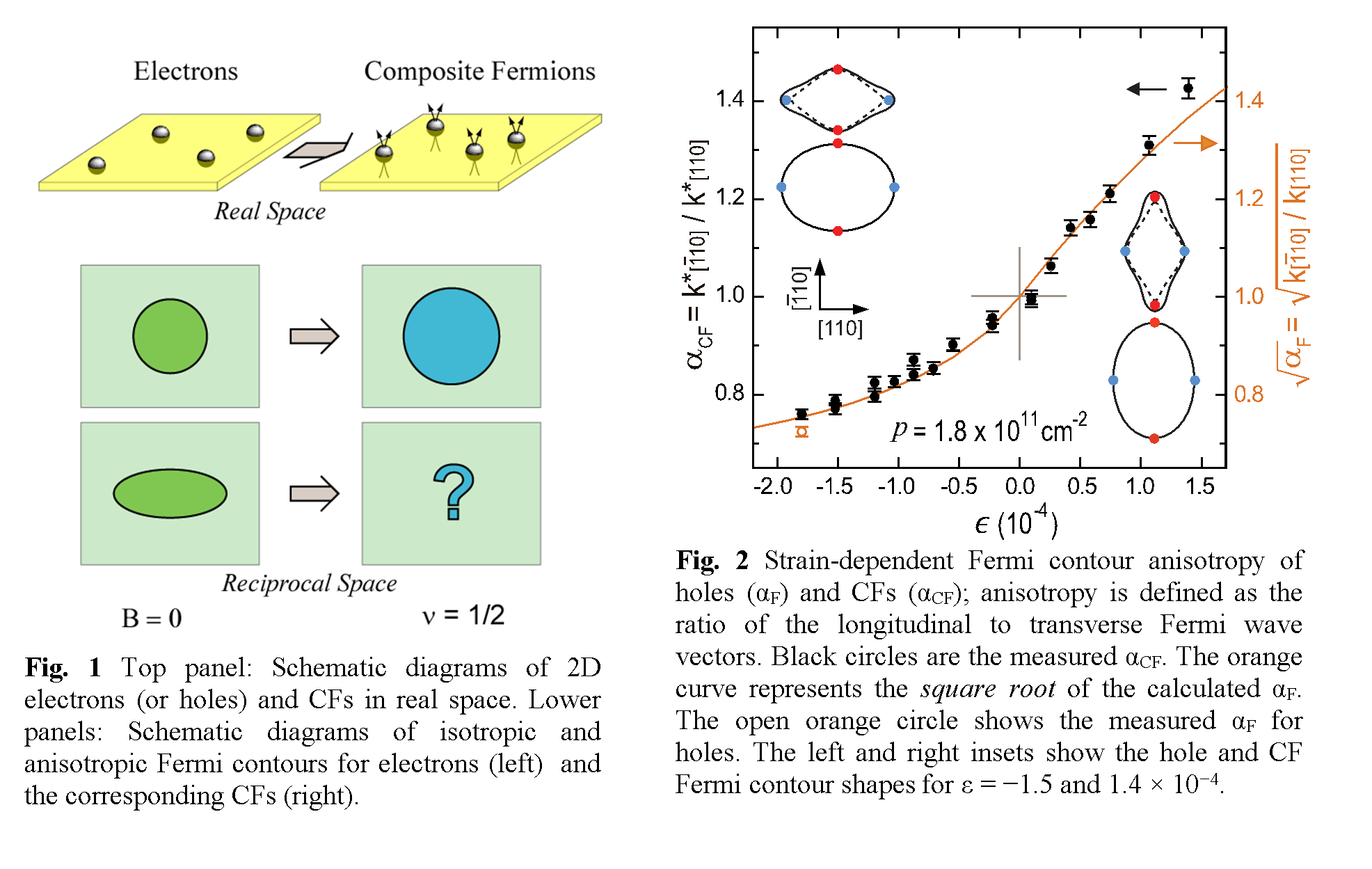
Direct Observation of Composite Fermions and Their Fully-Spin-Polarized Fermi Sea near ˝ = 5/2
The enigmatic even-denominator fractional quantum Hall state at Landau level filling factor ˝ = 5/2 is arguably the most promising candidate for harboring Majorana quasiparticles with non-Abelian statistics and, thus, of potential use for topological quantum computing. The theoretical description of the ˝ = 5/2 state is generally believed to involve a topological p-wave pairing of fully-spin-polarized composite fermions through their condensation into a non-Abelian Moore-Read Pfaffian state. There is, however, no direct and conclusive experimental evidence for the existence of composite fermions near ˝ = 5/2 or for an underlying fully-spin-polarized Fermi sea. In our study, we reported the observation of composite fermions very near ˝ = 5/2 through geometric resonance measurements and found that the measured Fermi wave vector provides direct demonstration of a Fermi sea with full spin polarization. This lends crucial credence to the model of 5/2 fractional quantum Hall effect as a topological p-wave paired state of composite fermions.
[Ref.: Hossain, Phys. Rev. Lett. 120, 256601 (2017), Editor’s Suggestion]
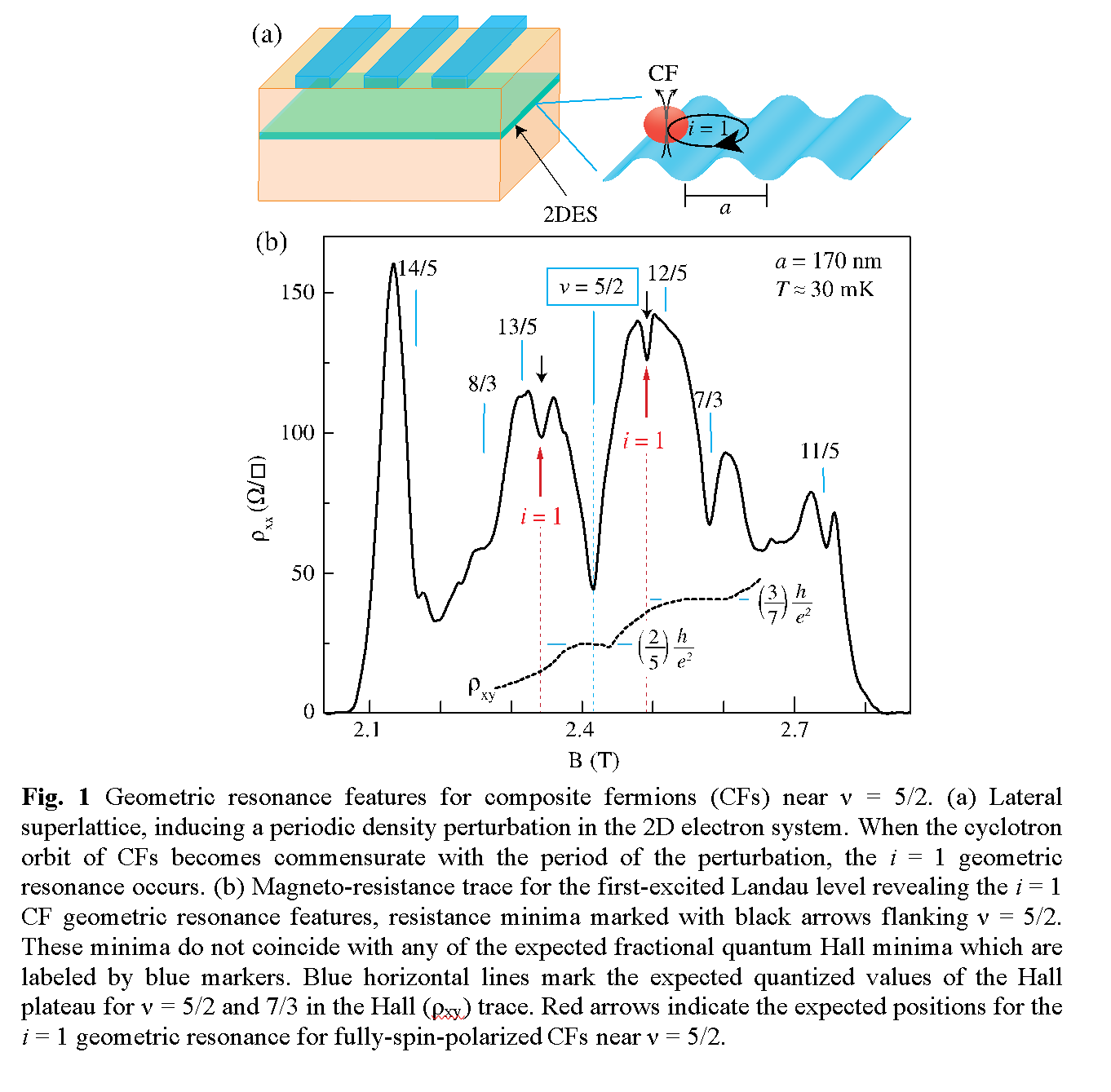
Realization of a “valley-superlattice” in AlAs 2D electron systems
In a number of widely studied materials, such as Si, AlAs, Bi, graphene, MoS2, and many transition metal dichalcogenide monolayers, electrons acquire an additional, spinlike degree of freedom at the degenerate conduction band minima, also known as “valleys.” External symmetry-breaking fields such as mechanical strain, or electric or magnetic fields, can tune the valley polarization of these materials, making them suitable candidates for “valleytronics.” Here we studied a quantum well of AlAs, where the 2D electrons reside in two energetically degenerate valleys. By fabricating a strain-inducing grating on the sample surface, we engineered a spatial modulation of the electron population in different valleys, i.e., a “valley superlattice” in the quantum well plane. Our results establish a novel manipulation technique of the valley degree of freedom, paving the way to realizing a valley-selective layered structure in multivalley materials, with potential application in valleytronics.
[Ref.: Mueed, Phys. Rev. Lett. 121, 036802 (2018), Editor’s Suggestion]
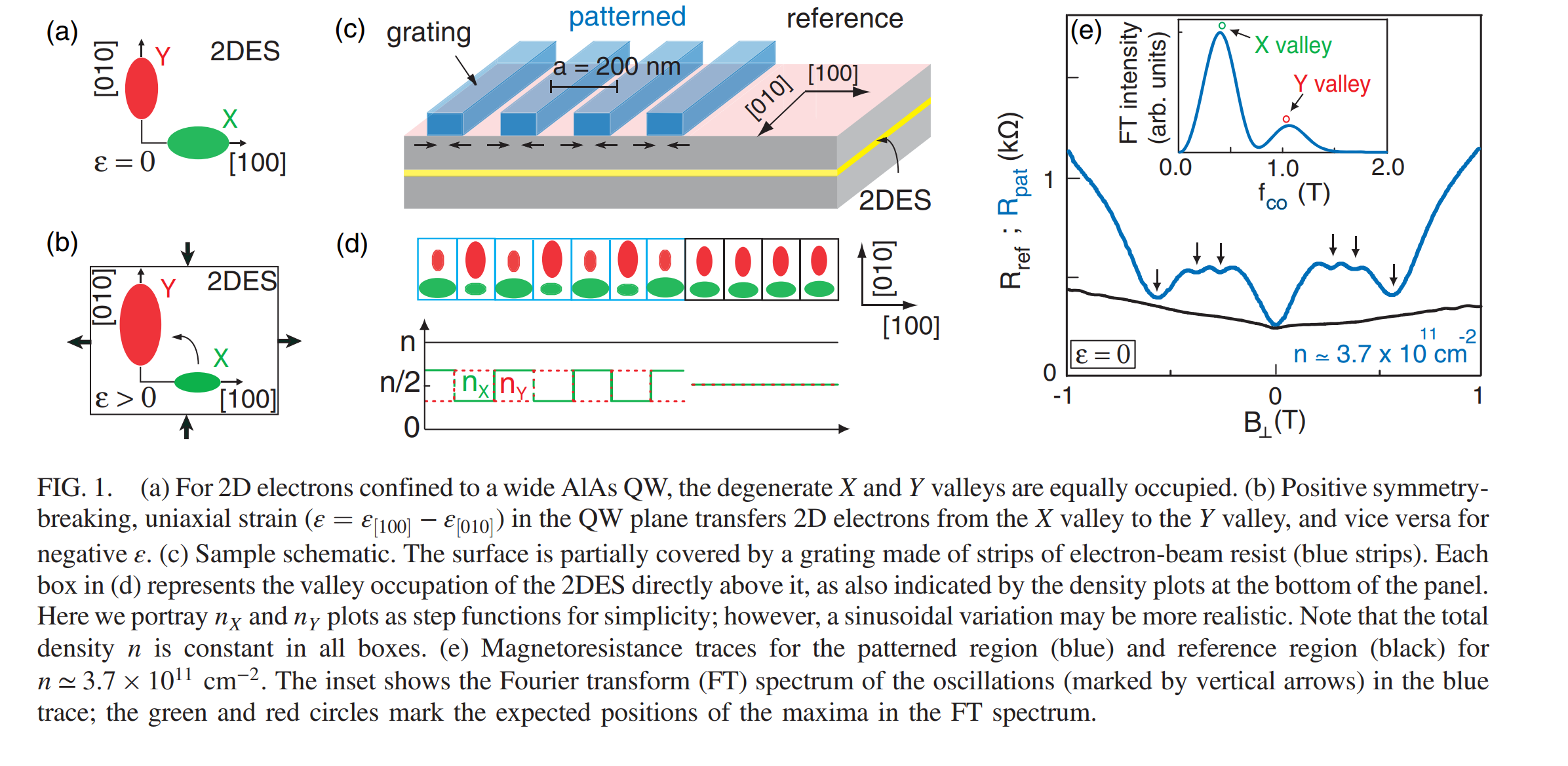
Berry's phase in a 2D hole system under stain
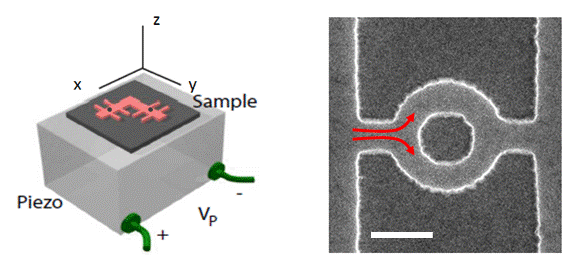
We study the Aharonov-Bohm effect in a 2D hole system under stain. Due to strong spin-orbit coupling, the signature of spin Berry phase can be observed in the resistance oscillations across the ring. We investigate the effect of strain on the Berry phase of spins that traverse the mesoscopic ring structure.
Aharonov-Bohm effect is a manifestation of wave-like nature of charged particles that traverse two different paths, resulting in oscillations of resistance as a function of magnetic flux that are enclosed by the mesoscopic ring structure. The resistance oscillations can be understood as the result of two interfering coherent electron waves that acquire phase difference while travelling two different paths. Interestingly, in a system with strong spin-orbit coupling, the charge wave function is also coupled with the spin degree of freedom. This spin related phase factor produces the additional beating structure in AB oscillation signals. It was found that the beating pattern was associated with the geometrical phase of spin, or spin Berry phase. In this project, we use strain to manipulate the spin geometric phase in the AB oscillations. By employing piezo-driven uniaxial strain we can tune the strength of spin-orbit coupling, which results in the spin-splitting by the effective in-plane field. We have demonstrated previously that strain can effectively modify the spin-split bands in the 2D hole system. The use of strain for spin manipulation in nanostructures possesses a great potential for future spin interference and quantum information devices.